The Reasons We Age – Hallmarks of Aging
Normal metabolism is an intricate network of biochemical processes, and the byproducts of these processes chronically damage our cells and essential biomolecules. Biological aging results from the accumulation of this cellular and molecular damage, which leads to the progressive loss of function and resilience in the tissues of aging people.
Causal risk factors like smoking, alcohol consumption, obesity, and inactivity cause additional damage to biological structures over and above those dictated by normal metabolism. The kinds of damage that specifically affect a given tissue lead to the many particular diseases of aging (such as cardiovascular disease, cancer, Alzheimer’s, Parkinson’s, and the rest), as well as many age-related functional limitations that are not classified as “diseases.”
Because the biology of aging drives age-related ill health, geroscience seeks to develop “longevity therapeutics” that target the biology of aging in order to maintain function and delay, prevent, or reverse multiple particular age-related diseases simultaneously.
Although it was long assumed that biological aging is a single, unified process, it is now widely understood that a number of important changes that happen as an organism ages are distinct processes and are potential targets for longevity therapeutics. One of the most widely accepted classification systems for these changes is the Hallmarks of Aging, a 2013 publication that identified nine unique categories (hallmarks) of age changes and illustrated how these categories interact to contribute to the emergence of age-related illnesses [1].
For years, these nine hallmarks of aging were used as the foundation for understanding aging and a framework to categorize the processes involed. But nothing stands still in science for long. New discoveries drive things forever onwards, and it is the same for geroscience and our understanding of aging. The nine hallmarks of aging were well established in the research community, but recently, some additional hallmarks have been proposed.
The 2023 paper “Hallmarks of aging: An expanding universe”, written by the original authors of the Hallmarks of Aging, proposes additional hallmarks based on increased understanding since its publication [2]. While these new hallmarks have just been introduced, they are included here in the interests of accuracy. A number of companies working in this space are already targeting these new hallmarks.
Why do we need the hallmarks of aging?
The hallmarks essentially serve as a framework to give researchers insights into how they might directly intervene against these aging processes to prevent age-related diseases. They also represent an important step towards scientific consensus on what aging is and moves us closer as a field towards a general theory of aging.
While the hallmarks are the most widely recognized way to categorize aging processes as we currently understand them, it is likely that they are not the full picture. Science is constantly evolving as new information comes in, and it will be the same for how we understand aging. However, they do reflect current levels of understanding, and this infographic summarizes what the hallmarks of aging are and how they are harmful.
Aging isn’t a disease, but the biology of aging leads to age-related diseases
Whether biological aging should be characterized as “a disease” has been subject to considerable debate among scientists. Both sides of the debate celebrate the fact that people are living longer in calendar years (“chronological aging”) but recognize that the biology of aging leads to the many individual diseases that are associated with a longer life today.
The promise of geroscience is to intervene in the biology of aging to decouple living longer from suffering diseases like cancer, heart disease, Parkinson’s, and Alzheimer’s, as well as other age-related debility, including loss of muscle strength, poor sleep, and a weakened immune system.
This is ultimately the goal of geroscience, to tackle age-related ill health at its root: the biology of aging. By ensuring tissues and organs remain biologically younger, longer and healthier lives will be possible in the future.
The hallmarks of aging in detail
The entire collection of DNA in an organism is known as its genome. In humans, almost every cell contains a copy of 3 billion base pairs that constitute the human genome. Each of our cells holds all the information necessary to reconstruct the human body.
A gene is a segment of DNA that produces a particular protein or a group of proteins. Typically, a human gene may vary by one to three base pairs among individuals. These variations can influence the structure and function of a protein, as well as how much, when, or where a protein is made.
These differences result in visible traits such as hair and eye color, as well as unseen factors like disease susceptibility and responses to medication. This explains why alterations to the DNA, even a single base pair change, can lead to significant and far-reaching effects on health.
Genomic instability refers to the occurrence of a high number of mutations in a genome and is caused by DNA damage that isn’t repaired. Genomic instability is a characteristic of most cancer cells and is predominantly associated with that disease.
The stability of DNA is under constant threat from external physical, chemical, and biological agents. It also faces internal dangers like replication errors, DNA strand breaks, and reactive oxygen species.
Unrepaired damage to DNA can disrupt critical genes and the mechanisms required to interpret and utilize genetic information, leading to dysfunctional cells. If these dysfunctional cells are not destroyed, then cancer is the most common consequence.
As cells divide, the protective caps called telomeres on the ends of our chromosomes get shorter. The enzyme telomerase can add repeat sequences to the telomeres, but in most cells, that isn’t enough to keep pace with the rate of loss.
Ultimately, the telomeres become critically short, telomerase production ceases, and the cell can no longer divide. At this point, the cell becomes senescent and under normal circumstances self-destructs via a process called apoptosis or is disposed of by the immune system.
Telomere attrition ensures that cells do not divide uncontrollably and is a safety measure against cells becoming cancerous. In simple terms, cells divide many times and along the way they pick up increasing amounts of damage to their DNA. Heavily damaged cells are dangerous, so telomeres work like a timer to remove the cell from circulation before it becomes too damaged.
Unfortunately, this safety feature is a double-edged sword. While it protects us against cancer, it also leads to tissue and organ dysfunction and influences other hallmarks of aging, such as epigenetic alterations, to support the onset of various age-related diseases.
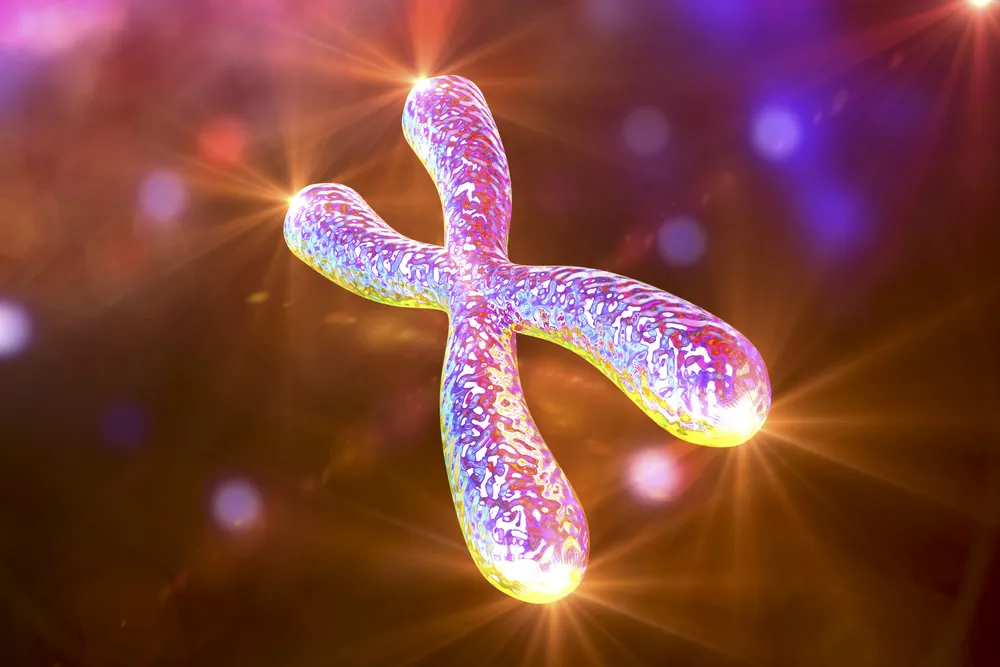
Epigenetics refers to how cells read the genetic code within the genome. Various epigenetic changes, such as DNA methylation, modifications of histones after translation, and chromatin restructuring, influence which genes are expressed or silenced.
At their core, epigenetic alterations are genetic modifications that change gene activity without changing the DNA sequence. Epigenetics is also why every cell in our bodies have the same genetic code, but different regions of DNA are turned off and on in each one, allowing us to have many unique cell types.
As we age, external factors like environmental pollution and toxin exposure and personal lifestyle choices, including smoking, excessive drinking, poor diet and a lack of exercise, can harmfully alter our epigenetics.
Age-related changes to epigenetics appear to make cells perform less efficiently. These changes appear to be reversible using a technique known as partial cellular reprogramming. Doing so appears to revert cells back to a functionally younger state. It is the hope that partial cellular reprogramming can be used directly to effectively “reset” aging cells. In recent years, researchers have found that biological age can be measured using these epigenetic changes.
In particular, DNA methylation can offer a detailed insight into a person’s overall well-being and health. Measuring DNA methylation can give a picture of how fast or slowly someone is aging and indicate how healthy that person’s lifestyle is [3].
This has led to epigenetic patterns being used as an “aging clock”. There are now several of these clocks in use within the research community, each looking at different sets of gene expression patterns. In fact, there are now companies offering epigenetic testing kits to the public with which to measure biological age.
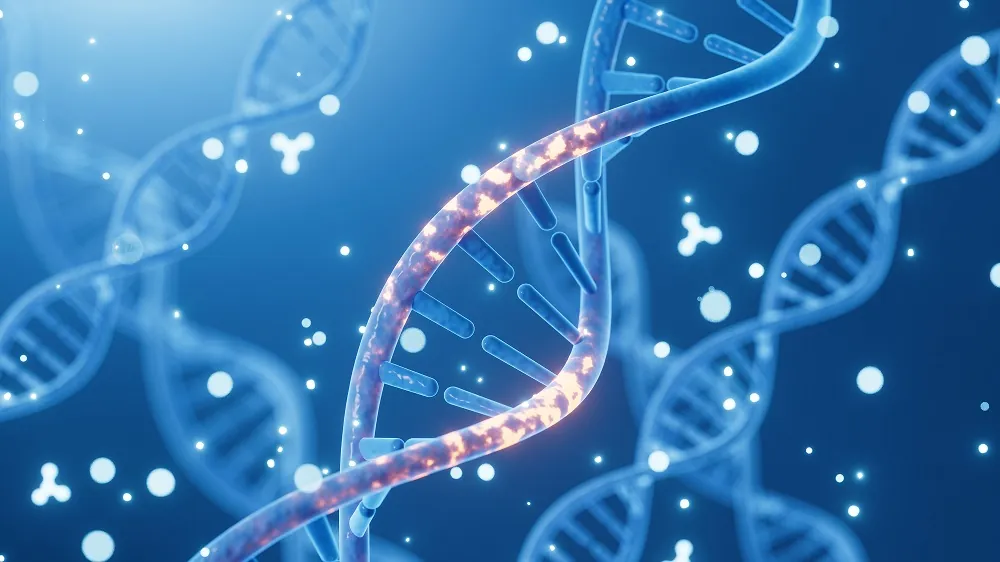
Proteins are responsible for a myriad of tasks within the body. Proteins do the bulk of the work within our cells and are needed to ensure the structure, function, and regulation of the body’s tissues and organs. Proteins are made up of hundreds to thousands of smaller parts called amino acids that are joined together in long chains.
In order for the proteins to perform their intended function, they must be shaped properly. If they are misfolded, the instructions they carry to other parts of the cell or to other cells will not be interpreted correctly. This process of protein creation, folding, and disposal is known as proteostasis.
Proteins that are damaged, misfolded, or no longer needed are tagged with a protein called ubiquitin. These marked proteins are then sent to the proteasome, a cellular machine that disintegrates unwanted proteins, to be broken down. Autophagy is another important degradation pathway for breaking down aggregates and damaged organelles. Unfortunately, as we age, the production, folding, and disposal of these proteins starts to fail, and the loss of proteostasis occurs.
If unfolded proteins are not properly refolded or broken down, they can accumulate and clump together, much like garbage piling up, causing toxic levels of proteins. This can lead to conditions such as Alzheimer’s and Parkinson’s.
Our bodies are complex machines, and the regulation of metabolism is a balancing act. There are four primary nutrient-sensing pathways that regulate metabolism [4].
- The insulin and insulin-like growth factor (IIS) pathway
- Mechanistic target of rapamycin (mTOR)
- The sirtuins
- AMP-activated protein kinase (AMPK)
These pathways are called “nutrient-sensing” due to their regulation by nutrient levels. Disruption to these pathways may cause metabolic disorders and hasten the aging process.
The IIS pathway is a key regulator of growth, metabolism, and aging, and integrates insulin and insulin-like growth factor 1 (IGF-1) signals. IGF-1, a hormone structurally similar to insulin, is primarily involved in growth and development.
The mechanistic target of rapamycin (mTOR) plays a key role in overseeing cell growth, metabolism, and aging. It comprises two separate complexes, mTORC1 and mTORC2, that react to nutrient levels, especially amino acids, influencing protein production, autophagy, and fat metabolism [5].
Sirtuins are a family of proteins that play a crucial role in regulating cellular metabolism, genome stability, and cell survival [6-7]. They rely on nicotinamide adenine dinucleotide (NAD+), a coenzyme that is critical in metabolism. NAD+ levels are typically higher during periods of low energy such as during fasting or hunger, which activates the sirtuins. When activated the sirtuins promote survival mechanisms to help cells survive during periods of nutrient scarcity.
AMPK serves as a key regulator of energy balance within cells. Its activation occurs when NAD+ levels rise, signaling a state of low energy [8]. This enzyme promotes catabolic processes that produce adenosine triphosphate (ATP), a universal energy source for cells, by facilitating glucose absorption and the breakdown of fatty acids. Additionally, AMPK inhibits energy-consuming activities such as protein synthesis and gluconeogenesis, functioning as a protective mechanism in response to energy scarcity.
Conditions like Type 2 diabetes and obesity are some of the consequences of deregulated nutrient sensing.
The mitochondria are important organelles of the cell. They break down food molecules and produce ATP, the molecular fuel of the cell. This is why they are commonly referred to as the power stations of the cell. They convert the food we eat into usable energy in the form of ATP [9].
Remarkably, mitochondria are likely not originally part of our cells; instead, they are thought to be stowaways and have DNA separate from our own. It is commonly believed that they fused with an ancient ancestor of all multicellular life, establishing a mutually beneficial partnership [10]. In return for producing energy for complex cellular functions, the mitochondria get nutrients and protection by being inside the cell.
One side effect of mitochondria producing energy is pollution, much the same as a real power station creates. In the case of the mitochondria, this pollution comes in the form of harmful reactive oxygen species (ROS), which bounce around the inside of the cell, potentially damaging the DNA and mitochondrial DNA when striking it.
This damage can cause mutations and contributes to genomic instability [11-13]. Therefore, damaged mitochondria can contribute to the risk of cancer.
Unfortunately, like many things in aging, our mitochondria become increasingly dysfunctional and less efficient at energy production and get increasingly damaged. They also stop creating new mitochondria quickly enough to replace losses, meaning that there is even less energy to go around.
Another consequence of mitochondrial dysfunction is that the mitochondria becomes less efficient in producing ATP and more likely to increase the amount of ROS they puts out. In this way, it becomes a vicious circle where more ROS is created and less energy is made available.
This loss of energy can even support the loss of proteostasis [14], drive muscle weakness [15], and contribute to levels of chronic background inflammation [16].
Cellular senescence refers to a permanent halt in the cell cycle, accompanied by noticeable changes in cell characteristics, especially the release of pro-inflammatory proteins known as cytokines.
This process can be initiated when cells reach the Hayflick limit, which is determined by the shortening of telomeres, but it may also be activated by various age-related factors such as DNA damage. The goal of this cell cycle arrest is to stop the replication of damaged and defective cells and to promote their eventual elimination.
Unfortunately, what is intended as a safety mechanism to reduce the risk of damaged cells becoming cancerous proves to be a double-edged sword as we grow older.
Normally, old and damaged cells destroy themselves through a self-destruct process called apoptosis. The immune system can also remove senescent cells that are damaged.
However, as we get older, more and more cells evade apoptosis and because the immune system declines, an increasing number of senescent cells remain in place. They no longer divide, and they also secrete a cocktail of inflammatory proteins that reduce tissue repair, can turn other nearby healthy cells senescent, and significantly contribute to aging [17, 18]. The more senescent cells that accumulate, the more they drive chronic inflammation and set the stage for multiple age-related diseases.
Once cells have reached their replicative limit, determined by telomeres, or they have become damaged, they need to be disposed of via apoptosis. Clearing away these old cells is an important part of keeping tissues and organs healthy. However, while removing these cells is vital, those losses need to be replaced with fresh new cells, which is where our stem cells come in.
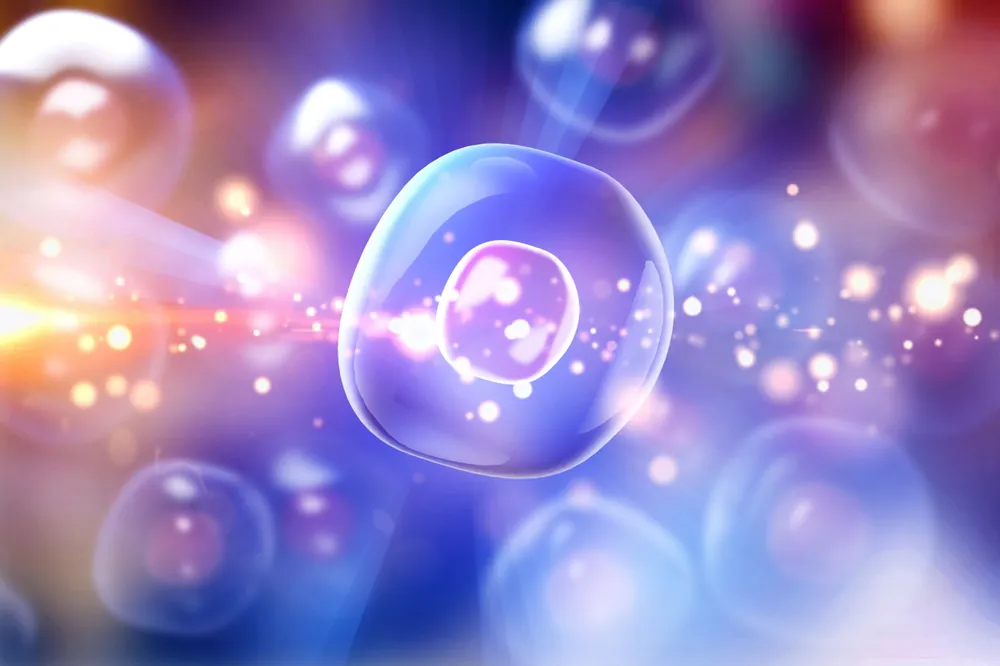
Normal cells have limited ability to modify their epigenetic configurations, while stem cells possess greater flexibility, enabling them to differentiate into nearly any cell type within the body. Stem cells undertake various roles, such as promoting signaling that enhances tissue function, regulation, and overall health [19].
Unfortunately, as we age, there is a reduction in stem cell activity, known as stem cell exhaustion, this can lead to impaired tissue and organ regeneration, support the onset of various age-related diseases, and weaken the immune system.
Similar to ordinary cells, stem cells are also affected by the buildup of DNA damage, loss of proteostasis, and mitochondrial dysfunction. Chronic levels of inflammation can also suppress the activity of stem cells too, meaning that instead of replacing cell losses they remain dormant.
Coordinating a highly complex machine like the human body is no easy task and it requires communication. Our cells communicate with each other, not just locally but to distant parts of the body too. It is this cell to cell communication that allows our biology to orchestrate all the many things that it does, no small feat.
Of course, aging impacts various aspects of our body, including how cells communicate with one another. As time progresses, the signaling environment throughout the body often becomes increasingly inflammatory, hindering effective communication between cells.
Increasing numbers of senescent cells that have evaded apoptosis, the failing immune system, harmful changes to gut bacteria, and more sources all drive inflammation and cause it to become chronic.
This chronic background of inflammation inhibits the immune system and potentially causes muscle wasting, bone loss, and other harmful effects. This altered intercellular communication leads to all kinds of coordination problems between different tissues and organs.
The gut is populated by a combination of bacteria, archaea, and viruses, often collectively referred to as the gut microbiota. The gut microbiota evolved in a symbiotic relationship with their human host. The microbiota are also unique to each individual and influenced by genetics, ethnicity, dietary factors, lifestyle habits, and environmental conditions.
They are an essential part of our biology and support many physiological functions like the digestion of nutrients, protection against pathogens, and the production of essential metabolites like vitamins. They also help maintain the integrity of our gut lining and protect us from conditions such as obesity, cancer, and heart disease.
The gut microbiota also communicate with both the peripheral and central nervous systems as well as various organs, significantly influencing overall health and well-being. If the communication between microbiota and the host is disrupted due to an imbalance or change to the microbiota or metabolism, then dysbiosis occurs.
Research suggests that individuals who live longer often possess fewer bacteria such as Bacteroides and Roseburia, while exhibiting a higher presence of other bacterial types like Bifidobacterium and Akkermansia [20]. In addition, another study showed that longer-lived people tend to have a higher presence of certain bacteria associated with health [21].
Autophagy is an important cellular recycling system that is known to slow with aging. Essentially, autophagy includes a number of processes that dispose of cellular waste and recycles unwanted cellular components such as proteins. Autophagy is quality control for cells.
Macroautophagy is a particular type of autophagy that involves trapping unwanted materials inside a small cellular container called a vesicle to be later digested by the lysosomes, the garbage disposal system of the cell.
Aging leads to disabled macroautophagy, which leads to the buildup of cellular waste, such as protein clumps, faulty organelles, and pathogens. Additionally, a decline in macroautophagy may increase inflammation, since proteins that trigger it are not being cleared away.
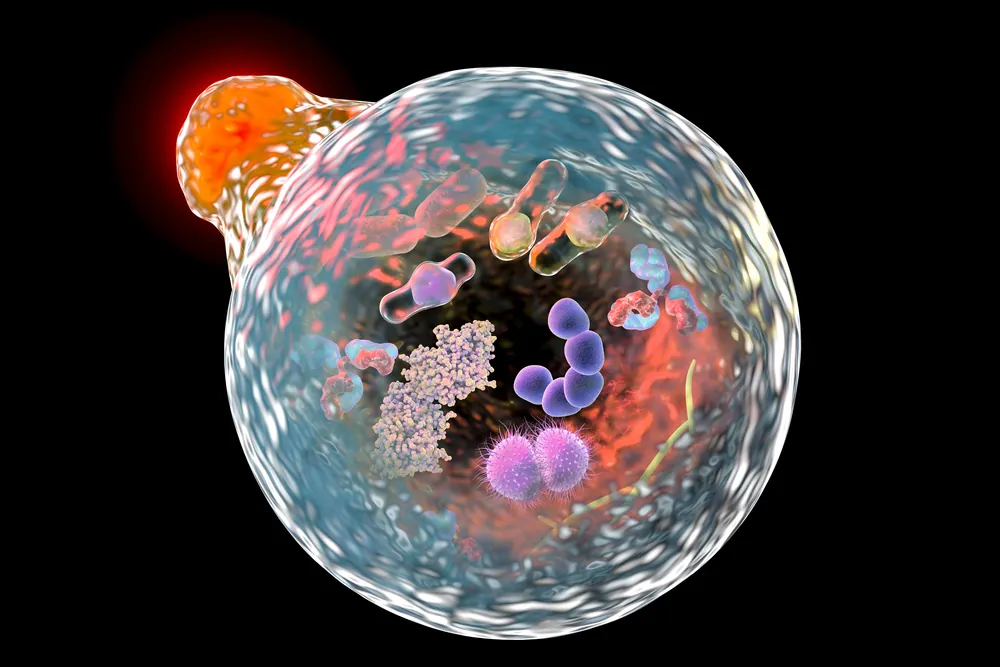
Chronic inflammation, what some researchers call inflammaging, is a very common consequence of aging. A common symptom of this smoldering background of persistent inflammation is swelling or stiffness of the joints. Research suggests that one in four American adults aged 45-64, and around half of Americans over 65, are diagnosed with osteoarthritis [22].
Under normal conditions, inflammation is an important defense mechanism. The immune system uses inflammation as a response to pathogens and toxins in order to fight back. This short-term inflammation is a useful thing; however, the problems begin when it becomes chronic.
The accumulation of senescent cells, cell debris, decline of the immune system, changes to gut microbiota, and many more things all contribute to chronic inflammation.
Persistent inflammation opens the door for a number of age-related diseases as well as interacts with the other hallmarks, making them worse. It can disrupt cellular function and communication, reduce tissue repair, and make the immune system dysfunctional. Neuroinflammation, intervertebral disc degeneration, arthritis, and osteoarthritis are conditions to which chronic inflammation can contribute.
Literature
[1] López-Otín, C., Blasco, M. A., Partridge, L., Serrano, M., & Kroemer, G. (2013). The hallmarks of aging. Cell, 153(6), 1194-1217.
[2] López-Otín, C., Blasco, M. A., Partridge, L., Serrano, M., & Kroemer, G. (2023). Hallmarks of aging: An expanding universe. Cell, 186(2), 243-278.
[3] Klemp, I., Hoffmann, A., Müller, L., Hagemann, T., Horn, K., Rohde‐Zimmermann, K., … & Keller, M. (2022). DNA methylation patterns reflect individual’s lifestyle independent of obesity. Clinical and Translational Medicine, 12(6), e851.
[4] Efeyan, A.; Comb, W.C.; Sabatini, D.M. Nutrient-Sensing Mechanisms and Pathways. Nature 2015, 517, 302–310.
[5] Laplante, M.; Sabatini, D.M. MTOR Signaling in Growth Control and Disease. Cell 2012, 149, 274–293.
[6] Imai, S. ichiro; Guarente, L. NAD+ and Sirtuins in Aging and Disease. Trends Cell Biol 2014, 24, 464–471.
[7] Sugishita, Y.; Suzuki-Takahashi, Y.; Yudoh, K. Nicotinamide Adenine Dinucleotide (NAD)-Dependent Protein Deacetylase, Sirtuin, as a Biomarker of Healthy Life Expectancy: A Mini-Review. Curr Aging Sci 2024, 17.
[8] Penugurti, V.; Manne, R.K.; Bai, L.; Kant, R.; Lin, H.K. AMPK: The Energy Sensor at the Crossroads of Aging and Cancer. Semin Cancer Biol 2024, 106–107, 15–27.
[9] Nolfi-Donegan, D.; Braganza, A.; Shiva, S. Mitochondrial Electron Transport Chain: Oxidative Phosphorylation, Oxidant Production, and Methods of Measurement. Redox Biol 2020, 37, 101674.
[10] Gray, M.W.; Burger, G.; Lang, B.F. The Origin and Early Evolution of Mitochondria. Genome Biology 2001 2:6 2001, 2, 1–5.
[11] Lesnefsky, E.J.; Hoppel, C.L. Oxidative Phosphorylation and Aging. Ageing Res Rev 2006, 5, 402–433.
[12] Basu, A.K. DNA Damage, Mutagenesis and Cancer. Int J Mol Sci 2018, 19, 970.
[13] McAdam, E.; Brem, R.; Karran, P. Oxidative Stress-Induced Protein Damage Inhibits DNA Repair and Determines Mutation Risk and Therapeutic Efficacy. Mol Cancer Res 2016, 14, 612–622.
[14] Korovila, I.; Hugo, M.; Castro, J.P.; Weber, D.; Höhn, A.; Grune, T.; Jung, T. Proteostasis, Oxidative Stress and Aging. Redox Biol 2017, 13, 550–567.
[15] Alway, S.E.; Mohamed, J.S.; Myers, M.J. Mitochondria Initiate and Regulate Sarcopenia. Exerc Sport Sci Rev 2017, 45, 58–69.
[16] Rimessi, A.; Previati, M.; Nigro, F.; Wieckowski, M.R.; Pinton, P. Mitochondrial Reactive Oxygen Species and Inflammation: Molecular Mechanisms, Diseases and Promising Therapies. Int J Biochem Cell Biol 2016, 81, 281–293.
[17] Freund, A., Orjalo, A. V., Desprez, P. Y., & Campisi, J. (2010). Inflammatory networks during cellular senescence: causes and consequences. Trends in molecular medicine, 16(5), 238-246.
[18] Coppé, J. P., Desprez, P. Y., Krtolica, A., & Campisi, J. (2010). The senescence-associated secretory phenotype: the dark side of tumor suppression. Annual review of pathology, 5, 99.
[19] Madrigal, M., Rao, K. S., & Riordan, N. H. (2014). A review of therapeutic effects of mesenchymal stem cell secretions and induction of secretory modification by different culture methods. Journal of translational medicine, 12(1), 260.
[20] Barbour, K. E., Helmick, C. G., Boring, M., & Brady, T. J. (2017). Vital Signs: Prevalence of Doctor-Diagnosed Arthritis and Arthritis-Attributable Activity Limitation – United States, 2013-2015. MMWR. Morbidity and mortality weekly report, 66(9), 246–253.
[21] Biagi, E., Franceschi, C., Rampelli, S., Severgnini, M., Ostan, R., Turroni, S., Consolandi, C., Quercia, S., Scurti, M., Monti, D., Capri, M., Brigidi, P., & Candela, M. (2016). Gut Microbiota and Extreme Longevity. Current biology : CB, 26(11), 1480–1485.
[22] Zhang, X., Zhong, H., Li, Y., Shi, Z., Ren, H., Zhang, Z., Zhou, X., Tang, S., Han, X., Lin, Y., Yang, F., Wang, D., Fang, C., Fu, Z., Wang, L., Zhu, S., Hou, Y., Xu, X., Yang, H., Wang, J., … Ji, L. (2021). Sex- and age-related trajectories of the adult human gut microbiota shared across populations of different ethnicities. Nature aging, 1(1), 87–100.